The TS-CHEM program provides an easy-to-use
software environment in which to analyze contaminant plume transport, and includes
a comprehensive library
of more than 30 different analytical solutions to the advection-dispersion equation.
Each solution incorporates different capabilities, including how it represents the
contaminant source and how the plume interacts with the aquifer. For this post
in the Solution Library series, we will be focusing on the AT123D-AT family of
models published by Dan Burnell in 2012 which represents an updated version of
the original AT123D model suite developed by G.T. Yeh at the Oak Ridge National
Laboratory.
What is AT123D?
The original AT123D was a suite
of analytical model solutions that are used to simulate one-, two- and
three-dimensional transport in groundwater. The program is complex, and allows
for many different source configurations, including
- patch sources
- line sources
- point sources
- volume sources
In contrast to
many analytical plume models that represent the source using a first-type
specified concentration, AT123D represents the source as a second-type
specified mass flux boundary condition. This means, for example, if you know
the nitrogen load from a residential dwelling to a septic system (i.e. number
of residents times average daily nitrogen load per person) you can represent
that source in AT123D as milligrams of nitrogen per day instead of trying to
estimate a septic source concentration value. The primary difference between AT123D
and AT123D-AT is in how the solver arrives at a solution to the
advection-dispersion equation. AT123D-AT incorporates a Romberg numerical
integration scheme that works to prevent oscillations, speed convergence, and
improve accuracy for a wide range of input parameter combinations.
A conceptualization of the many
possible specified mass flux source geometries available in AT123D-AT is shown
in Figure 1 below, and can also be found in the Model Selection Tool in TS-CHEM:
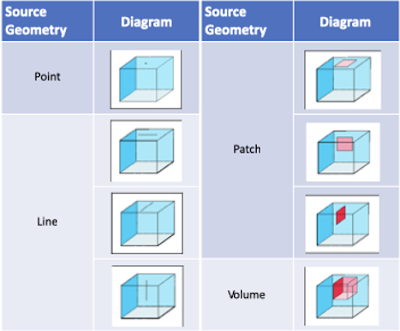 |
Figure 1 - AT123D-AT Source Geometries
|
TS-CHEM was developed with
usability in mind, so the numerous AT123D-AT solutions available to the user
(differing aquifer geometries, source geometries, and source types) have been
broken up into six “versions” (see table below). These pre-configured versions
allow the user to select the type of AT123D-AT model that will best represent
their site (aquifer and source), and to specify the desired parameter input
values to represent site-specific properties, using model types that closely
match conditions simulated by other analytical ADE solutions included in TS-CHEM’s Solution Library. This last consideration
makes it easier to compare different plume transport solutions (e.g. a
first-type source vs a second-type source) by selecting similar type models
(e.g. with aquifer boundaries or without aquifer boundaries) from the TS-CHEM Solution Library.
The following nomenclature is
used between each version to help the user quickly determine which version they
are looking for: Infinite boundary (I), Finite boundary (F), Constant mass
release rate (C), Instantaneous release source (I), Time-variable (transient)
mass flux (T):
Model
Version
|
Aquifer
Boundary
|
Source
Type
|
Analagous
TS-CHEM models
|
AT123D-AT IC
|
Infinite
unbounded
|
Constant mass
flux
|
3DADE-3 or
3DADE-4 (patch source)
|
AT123D-AT II
|
Infinite
unbounded
|
Initial mass
instantaneous release
|
3DADE-5 or
3DADE-6 (volume source)
|
AT123D-AT IT
|
Infinite
unbounded
|
Constant
specified concentration
|
ATRANS4 (with
concentration stepping)
|
AT123D-AT FC
|
Finite
bounded
|
Constant mass
flux
|
ATRANS1
|
AT123D-AT FI
|
Finite
bounded
|
Initial mass
instantaneous release
|
3DADE-5 or
3DADE-6 (volume source)
|
AT123D-AT FT
|
Finite
bounded
|
Constant
specified concentration
|
ATRANS4 (with
concentration stepping)
|
What are the differences
between the AT123D-AT models?
The two defining factors that highlight
the differences between the different AT123D-AT models are 1) whether the
aquifer extent is assumed to be infinite (as is the case for many analytical
transport solutions) or finite (bounded horizontally or vertically), and 2) how
the source concentration or flux is applied at the model boundary over time
(continuous, instantaneous pulse release, or time-varying). The three different
types of source concentration fluxes are conceptualized in Figure 2 below:
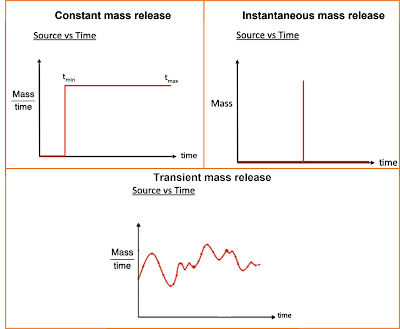 |
Figure 2 - AT123D-AT Model Source Types
|
As you can see in Figure 2, there
are three distinct source types. The source type that is most appropriate to
use in a particular application is dependent on the known conditions at a site,
and/or the known or assumed conditions of the source.
What applications are the AT123D
models best suited for?
As discussed in previous blog
posts, TS-CHEM can assist environmental professionals with the development of
conceptual site models (CSMs) that characterize the extent and behavior of
groundwater contaminant plumes. The AT123D-AT models are highly flexible, able to
provide users with the ability to evaluate solute fate and transport in one-,
two- or three-dimensions. In its fundamental form, AT123D-AT is solving the 3D
ADE equation in a 1D (X-direction only) aquifer flow field, but 2D plume
transport and even 1D “column” transport can be set up using the bounded
aquifer settings. i.e. the AT123D-AT “F” models (FC, FI, and FT) can be used to represent
sites where the aquifer thickness and/or width is known or is believed to be
bounded (finite aquifer
boundary). This type of AT123D-AT model is similar to the ATRANS
family of models which include an upper (water table) and lower (aquifer base)
no-mass-flux boundary.
But the AT123D-AT models are not
all limited to bounded aquifer conditions. The AT123D-AT “I” models (FI, II, and IT) can
be used to represent sites where there is no limitation on aquifer thickness or
width (infinite aquifer
boundary). This type of AT123D-AT model is similar to the 3DADE
family of models, which do not impose any finite boundaries on the aquifer.
Further, the investigator can use
a variety of patch source geometries to best represent the conditions at their
site. For example, if an investigator has information on source mass flux at
the downgradient edge of a source area, they may use a patch source. Or, if a
large regional scale model is being considered, the user may want to utilize a point source to track
the general plume behavior over time.
When choosing between source
types, a user may choose to use a constant mass release source AT123D-AT model If only a single source
concentration is known, and/or the investigator wished to perform a
conservative analysis in which the source does not deplete through time. If the
user wanted to investigate the plume behavior in a case where a slug of solute
is introduced into the groundwater, they can use an instantaneous release source type. If
information is available on the changing history (both increases and decreases)
of source flux over time, the transient source type can be used.
Similar to the ATRANS1 model, the AT123D-AT
FC model is useful for simulating scenarios that have aquifers of a finite
extent that can be represented by a continuous mass flux. This type of analysis
may be useful for evaluating a conservative maximum plume extent, or when the
plume becomes stable (Figure 3):
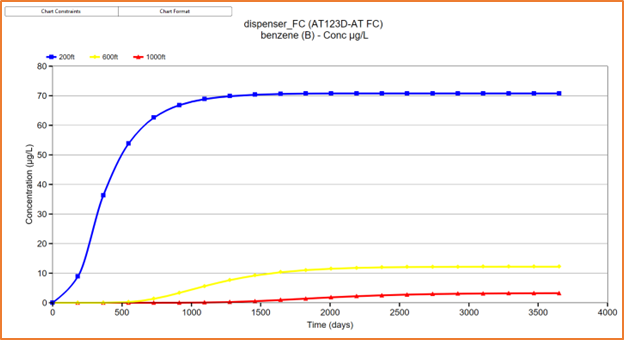 |
Figure 3 - AT123D-AT FC solution showing maximum plume extent for a stable benzene plume with a constant source flux of 0.001 ld/d at 200ft, 600ft and 1000ft from the source. |
The
AT123D-AT FI model is
useful for simulating scenarios where a slug of contaminant is assumed to have rapidly
entered the groundwater. This type of model is sometimes used in an emergency
spill response analysis if an investigator wants to simulate a conservative
condition where a “slug” of contaminant enters the groundwater instantaneously and
then is allowed to flush away from the spill area toward a possible receptor
location (Figure 4):
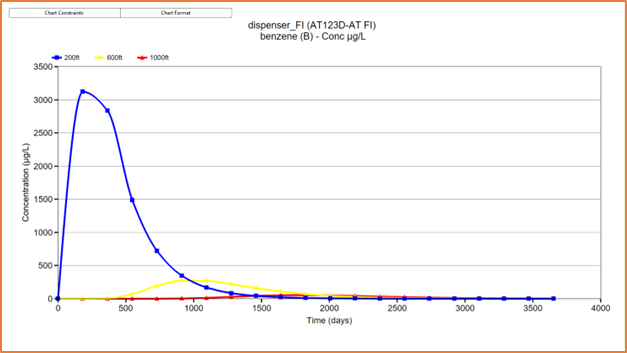 |
Figure 4 - AT123D-AT FI solution showing plume concentrations up to 4000 days after an instantaneous mass input of 22.05 lbs of benzene introduced to groundwater. Concentrations are shown at 200ft, 600ft and 1000ft from the source. |
Similar to the ATRANS4 model, the AT123D-AT
FT model allows the user to define transient source behavior. Unlike
ATRANS4, the AT123D-AT FT model uses a second-type boundary condition and is
therefore defined by time-flux pairs (instead of time-concentration pairs). The
transient source condition allows the user great flexibility in how they define
the time series of their source and can enable investigators to simulate
complex scenarios where there may be intermittent single sources, multiple
sources that overlap at different times, or even source termination that would
result from remedial actions.
An example of a time-variable
source flux is shown in Figure 5 below for a hypothetical release from an
underground storage tank (UST). The benzene source progressively decreases
until it eventually reaches zero after 2000 days. This might be indicative of a
UST that leaks over time, or it could be indicative of a UST removal at around
500 days with a small amount of product remaining after removal. In either
case, the benzene concentration is reduced over time through degradation/natural
attenuation and flushing.
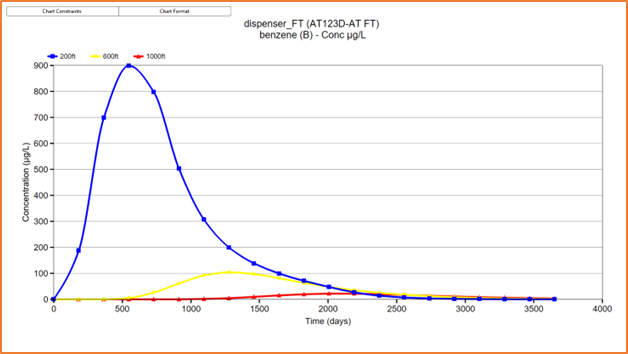 |
Figure 5 - AT123D-AT FT solution showing benzene plume concentrations entering groundwater from a hypothetical underground storage tank at 200ft, 600ft and 1000ft from the source. |
To summarize: the AT123D-AT
family of models available in the TS-CHEM Solution Library allow for a very flexible representation of the source
over space and through time and can be used for a variety of
environmental scenarios and conditions. The feature that sets the AT123D-AT
models apart is that they are the only models in the TS-CHEM solution library
that utilize a second-type (specified mass flux) source boundary condition,
allowing for users to directly input an estimate of source mass flux for their
site. AT123D-AT also differs from AT123D in that it incorporates enhanced
solver capabilities that reduce solve times and increase solution accuracy. The
six AT123D-AT model types included in TS-CHEM allow a user to easily identify which
model is best for their site or application. These models can assume either a
finite or infinite aquifer boundary and a multitude of source geometries and
source release types.
To learn more about TS-CHEM, or
to download a FREE DEMO
VERSION of the software, visit the TS-CHEM
Website today!